Research
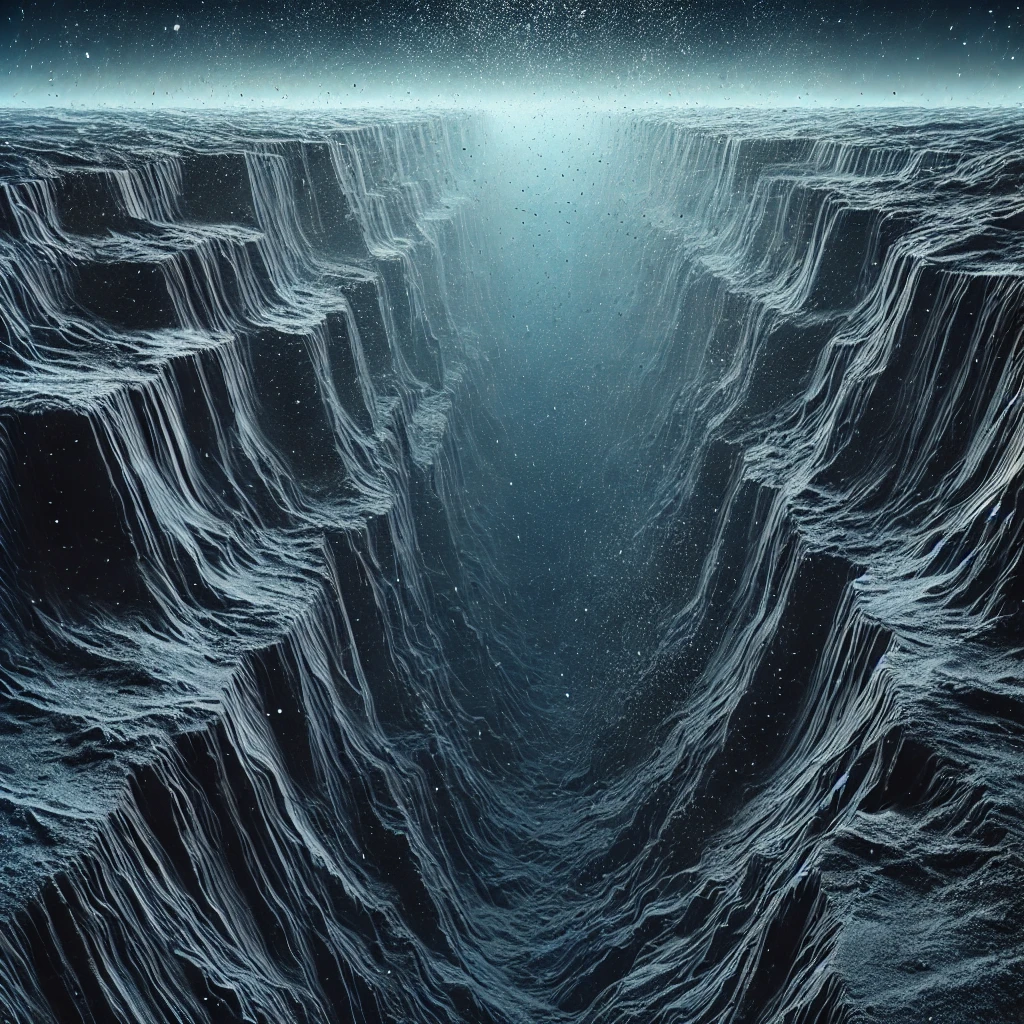
Abyssal Ocean Turbulence
The abyssal ocean is a dynamic environment where intense turbulence and complex mixing processes are driven by variations in temperature, salinity, and internal tides. Gravitational forces act on density differences in seawater, along with internal tides moving water along sloping seafloor topography. This interaction with rugged terrain generates turbulent eddies and bottom boundary layer turbulence, facilitating the exchange of heat, carbon, and nutrients between deep ocean layers and the surface. These exchanges play essential roles in marine ecosystems and climate regulation.
Boundary layer dynamics over sloping topography in stratified, rotating fluids are crucial for understanding how mixing, turbulence, and large-scale energy transport impact the distribution of heat, nutrients, and other tracers within Earth’s systems. Decades of research have revealed complex interactions among internal waves, stratification, and topographic effects, providing insights into boundary layer turbulence (BLT) and mixing mechanisms under tidal forces in stratified environments.
In abyssal circulation, dense, cold water forms near polar regions, sinking and spreading along the ocean floor toward the equator due to density gradients. This bottom boundary layer turbulence contributes to the global ocean overturning circulation, a system that redistributes heat and nutrients worldwide. It serves as a long-term sink for carbon and heat, influencing weather, marine ecosystem stability, and Earth’s climate.
With the rise of deep-sea mining, there is growing concern over its potential impact on abyssal turbulence and the ecosystems it supports. The deep-sea floor contains valuable resources like manganese nodules and cobalt-rich crusts essential for technology, but mining can disrupt sediment layers, increase turbidity, and alter natural mixing processes. This disruption threatens nutrient balance and deep-sea habitat health.
Research on abyssal turbulence and human impacts, such as deep-sea mining, is essential for preserving the deep ocean’s role in climate stability and biodiversity.
I study BLT using large eddy simulations of idealized BLT and aim to shed light on the underlying mechanisms that drive topographical mixing and upwelling, which are crucial in climate regulation.
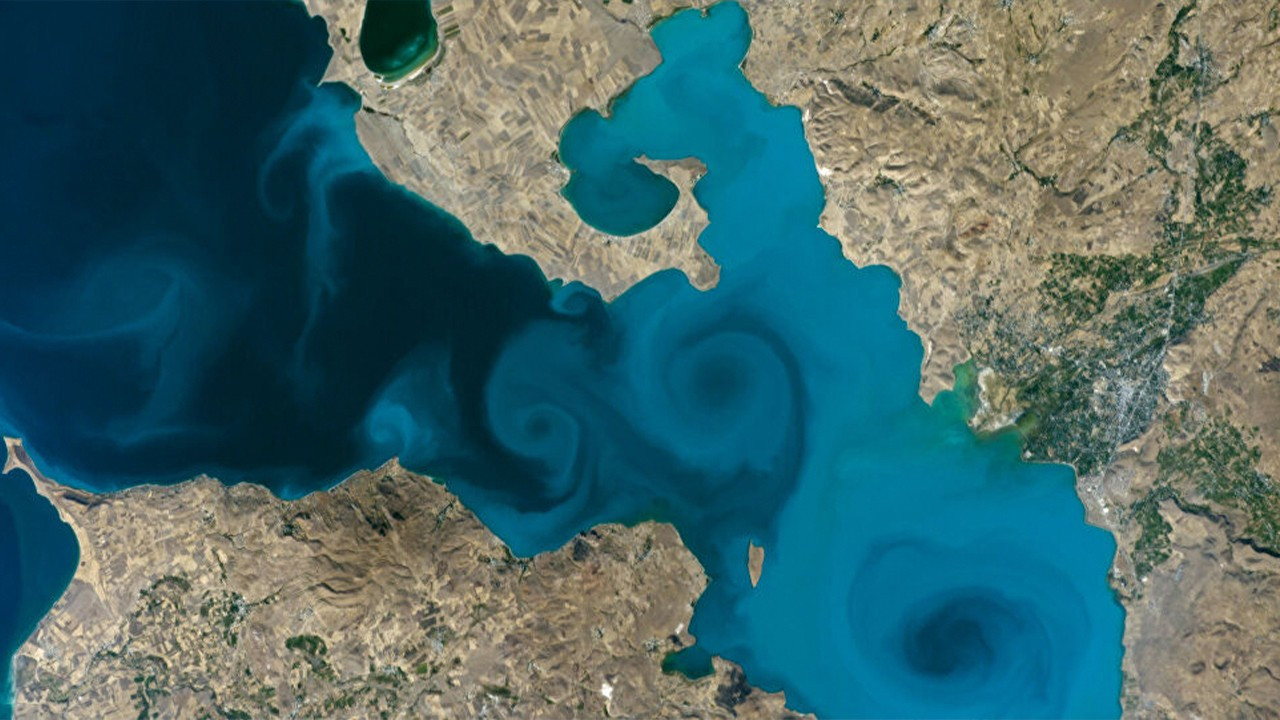
Mixing in Estuaries and Straits
In estuaries and straits, stably stratified exchange flows occur when two fluid layers of differing densities interact, with the denser layer settling below the lighter one due to gravitational forces, forming a stable density gradient. This layered stratification is critical for understanding fluid dynamics in natural and engineered systems, such as estuarine environments, building ventilation, and climate mitigation. Notably, estuaries and straits function as geophysical boundary layers, where distinct water masses meet and mix, forming localized gradients in salinity, temperature, and density. These gradients drive important mixing and transport processes, which regulate the movement of nutrients, sediments, and pollutants and impact broader geophysical systems.
A key model for studying such flows is the Stratified Inclined Duct (SID), where an exchange flow is sustained within a long, gently sloping duct. The SID model replicates continuously forced density-stratified flows commonly found in estuarine systems, providing insights into wave dynamics and transitions to turbulence.
Dynamics of Stratified Flow and Transition to Turbulence
Experiments in SID reveal that as the duct’s tilt angle increases, interfacial waves emerge and ultimately transition into turbulence, closely linked to a threshold in the exchange flow rate, as predicted by inviscid two-layer hydraulic theory. To explore these hydraulic mechanisms, recent studies use:
- Direct numerical simulations (DNS) to obtain comprehensive flow data across key regimes,
- Two-layer averaging of these simulations, and
- Inviscid two-layer shallow-water and instability models for analyzing interfacial wave behaviors.
In stable conditions, the flow is subcritical, laminar, and controlled at the duct’s boundaries. However, as tilt increases, the flow transitions to a supercritical state, becoming unstable and vulnerable to long-wave instabilities, progressing from stationary waves to wave breaking and intermittent turbulence at larger tilt angles.
The SID setup illustrates how coherent structures—organized regions within turbulent flows—arise amidst apparent randomness, driving energy transfer and mixing processes. In turbulent flows, these structures often appear as vortices, shear layers, and wave patterns, which enhance mixing by increasing the surface area over which diffusion occurs.
Coherent Structures and Turbulent Mixing in Stratified Flows
Coherent structures are essential in turbulent mixing as they organize chaotic motions and drive mixing efficiency across a wide range of scales. For example, vortices maintain rotational coherence from large to small scales, while shear layers, characterized by steep velocity gradients, can roll up into vortices due to instabilities. Kelvin-Helmholtz waves often develop across shear layers, and Tollmien-Schlichting waves emerge in boundary layers, further contributing to mixing. These structures, though intermittent, persist across flow regimes and can merge, split, and dissipate, enabling energy transfer from larger scales down to smaller dissipative scales.
The characteristics of coherent structures—intermittency, variability in scale, and persistence—have profound implications for mixing in stratified flows. Vortices, for instance, enhance mixing by stretching and folding scalar fields like temperature and concentration, creating extensive interfaces for diffusion. Understanding these structures improves predictions of mixing rates and patterns, such as pollutant dispersion, by capturing coherent dynamics within turbulence models like Large Eddy Simulation (LES) and Direct Numerical Simulation (DNS).
Practical Applications
This understanding of stratified flow dynamics and coherent structures has broad applications:
-
Estuarine and Coastal Management: Enhanced predictions of pollutant and nutrient dispersion in estuarine and coastal environments aid in ecological management. Accurate predictions of mixing rates are especially important for mitigating saltwater intrusion into freshwater sources and managing flooding events. In these scenarios, the rate at which saltwater mixes with freshwater determines the extent of intrusion and affects the salinity levels that can impact ecosystems, agriculture, and drinking water supplies.
-
Environmental Engineering: Insights into mixing processes are essential for environmental monitoring, especially for predicting the behavior of pollutants and nutrients in natural waters and the atmosphere.
Overall, the study of mixing in stratified estuarine and coastal flows, coupled with the influence of coherent turbulent structures, provides a comprehensive framework for addressing complex environmental and industrial challenges, advancing predictive capabilities in both natural and engineered systems.
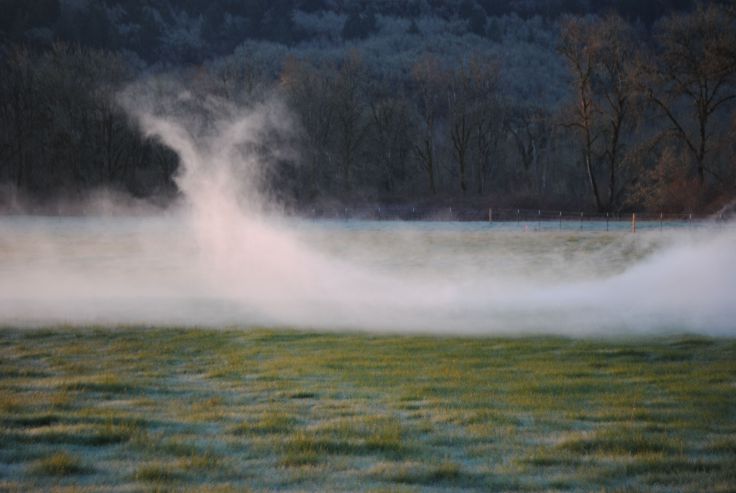
Atmospheric Boundary Layer Turbulence
Turbulence is a chaotic and powerful phenomenon in fluid dynamics, influencing everything from weather patterns to air quality. It’s what creates the swirling motion in clouds, drives ocean currents, and plays a key role in climate and pollution movement. My research focuses on a particular kind of turbulence called stably stratified turbulence, which occurs in layered environments like the atmosphere and oceans, affecting both natural processes and human activities.
What is Stably Stratified Turbulence?
Stably stratified turbulence happens when there’s a stable layering in fluids, like warm air sitting on top of cooler air. This stable layering, often due to temperature differences, prevents vertical mixing and keeps the air or water layers mostly separate. A key factor controlling this is the Richardson number (Ri), which is used to predict when turbulence will weaken or stop. When Ri is high (above a certain threshold), turbulence decreases, and the fluid layers remain intact.
In most types of turbulence, energy moves from large swirls down to smaller ones until it fades. But in stably stratified turbulence, this downward “cascade” is interrupted. Instead, energy often flows horizontally, forming layered, wave-like structures. My research examines how these layers generate internal gravity waves—oscillating motions that play a major role in energy transfer across these stable layers.
The Role of the Atmospheric Boundary Layer (ABL)
The atmospheric boundary layer (ABL) is the lowest part of the atmosphere, where the Earth’s surface directly influences the air above. It can stretch from a few hundred meters to a couple of kilometers, depending on the terrain, weather, and time of day. The ABL is critical for weather and climate because it’s where air masses mix, affecting everything from daily weather to long-term climate patterns. In stably stratified conditions—often occurring at night or under high-pressure systems—turbulence is much weaker, which changes how air mixes.
A key area of my research is the nocturnal boundary layer (NBL). After sunset, the ground cools, creating a temperature “inversion” where the air above is warmer than near the ground. This setup reduces mixing and traps pollutants close to the surface. In the NBL, turbulence tends to be intermittent, and the lack of vertical mixing sometimes leads to a low-level jet, a narrow band of fast-moving air that moves pollutants and heat horizontally.
Studying Stably Stratified Turbulence
To understand these processes, I use numerical models such as large-eddy simulations (LES) and direct numerical simulations (DNS). These models replicate buoyancy effects and layering dynamics, though resolving the fine details of wave-turbulence interactions remains computationally challenging.
Why This Research Matters
My research on stably stratified turbulence in the ABL is essential for several reasons:
-
Weather Forecasting: Accurately representing stable layers improves predictions for nighttime weather and stable high-pressure conditions.
-
Climate Modeling: Long-term climate models rely on a precise understanding of how stable stratification affects heat and moisture movement in the ABL.
-
Air Quality: During stable conditions, pollutants are often trapped near the surface, reducing air quality. Insights from my research help in predicting pollution behavior and improving mitigation strategies.
-
Aviation Safety: Internal gravity waves and turbulence in these stable layers can impact aviation. Enhanced models and forecasts improve flight safety under these conditions.